The emerging science of gravitational wave astronomy is optimistically named. Astronomy depends ultimately on observations, yet the only output of gravitational wave detectors has so far been noise generated within the instruments. There is good reason, based on experimental and theoretical progress, to believe that things are about to change. As an example of progress on the theoretical side, Kenta Kiuchi of Waseda University, Yuichiro Sekiguchi of the National Astronomical Observatory, Masaru Shibata of Kyoto University (all in Japan), and Keisuke Taniguchi of the University of Wisconsin, US, report in Physical Review Letters simulations of neutron star mergers that reveal new details of the gravitational waves they are expected to emit.[1]
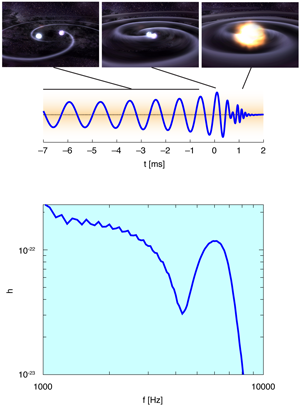
In parallel with the development of ground-based detectors, there has been substantial design progress for detectors in space. The principal example is LISA,[4] which received effusive endorsement from the National Academy of Sciences: "LISA is an extraordinarily original and technically bold mission concept. The first direct detection of low-frequency gravitational waves will be a momentous discovery, of the kind that wins Nobel Prizes".[5] Space-based detectors will not likely be making that low-frequency (<0.1 Hz) discovery for another ten years at least - not for lack of inherent sensitivity or progress in technology development, but rather because rapid deployment is not a characteristic of billion-dollar space research missions.
Meantime, the effort to improve ground-based detectors, which operate at higher frequency (10 to 10,000 Hz), is proceeding apace. What is the motivation compelling some of us to spend entire careers building telescopes that have yet to see the gravitational equivalent of first light? The thrall of zero is one conceivable answer. That is, the absence of signals at predicted levels places real constraints on astrophysical phenomena, and ultimately could test Einstein's theory of general relativity. But most of us would trade a thick stack of publications with "search for" in the title for a single thin "discovery of." It's not about zero. Rather, the case that LIGO and VIRGO are almost good enough to see signals stands up to scrutiny. This is not simple optimism: the detection prediction is derived from a synthesis of electromagnetic astronomy and astrophysical models of sources that seem inevitable.
For many years the favored source of gravitational waves for ground-based detectors has been the inspiral of a compact binary system consisting of one neutron star plus a companion that is either another neutron star or a black hole (Fig. 1, top). The orbital motion generates gravitational radiation at a frequency that chirps as the orbit decays and speeds up. The chirp waveform can be calculated accurately from a handful of parameters such as the masses and spins of the two stars and the inclination angle of the orbital plane. This waveform parameterization allows for coherent integration of the last minute or so of the life of the binary system, when the gravitational wave signal is strongest.
In the 1987 proposal to the National Science Foundation that first described the LIGO concept,[6] Kip Thorne had this to say about detection prospects in general: "The most certain of the sources is coalescence of neutron-star binaries: Estimates based on pulsar statistics in our own galaxy suggest that to see 3 such events per year one should look out to 100(+100/-40)Mpc distance. For supernovae the event rate is known to be roughly one each 40 years in our own galaxy and several per year in Virgo, but the amount of radiation emitted is very uncertain. For black hole births, both the wave-emission efficiency and the distance to which one must look are highly uncertain."
Since then, refined calculations of supernova strength have moved that source lower on the list, and the rate of black hole births remains uncertain. But estimates of the instrument range required to see a neutron star binary inspiral[7,8,9,10,11] has held fairly stable with the discovery of a few more galactic radio pulsars in binary orbits. A current estimate [9] is that the merger rate within the galaxy is 80(+200/-66)/Myr,[10] which at a galactic density of 10-2/Mpc3 corresponds to a required detector range of 44 to 120 Mpc - a somewhat more promising number than the 1987 estimate of 60 to 200 Mpc.
After the inspiral chirp comes the coalescence. The end stage of the neutron binary system is a complex explosion that takes as little as a few milliseconds: tidal disruption, core collapse, merger, and formation of the final-state black hole. The merger hypothesis holds that each short (0.1 - 1 s) gamma-ray burst observed by satellites is generated at the instant of merger, perhaps from shock waves formed as the neutron star collapses. This hypothesis was supported by the identification of several short bursts in 2005 as originating beyond the galaxy.[12] Longer gamma-ray bursts, those lasting more than 2 s, arise from different etragalactic events, including supernovae.
A recent search for gravitational wave inspiral signals in the LIGO and VIRGO detectors used short gamma-ray bursts detected by gamma-ray and x-ray satellites as timing triggers.[13] Analyzing data stretches preceding 22 distinct gamma-ray bursts, the result was no signal with 90% confidence from a neutron star/black hole binary within a median distance of 6.7 Mpc, or from a double neutron star system within 3.3 Mpc.
These numbers fall short of the range required for detection, but there is already data collection of higher sensitivity in progress, and further enhancements are planned after that run is finished. Beyond that, Advanced LIGO, with ten times the sensitivity and consequently ten times the range, should be in operation by 2015. The double neutron star inspiral range for Advanced LIGO is projected to be 300 Mpc,[14] adequate to see several events per year even at the pessimistic end of the source estimates. There is little doubt that the new window on the universe will finally be cracked open. Parameters derived from the waveform fit will constitute a rich vein of data: confirmation of the merger hypothesis, a survey of masses and spins of neutron stars, and, tantalizingly, a calibration-free measure of the source distance that can be used to measure dark energy.
The results of Kiuchi and colleagues look beyond even Advanced LIGO. The authors present one of the first detailed simulations of the waves generated by the merger. Figure 1 shows their simulation result for the precursor chirp and the merger itself starting at t=0 milliseconds. The chaotic-looking merger waveform contains information that is completely inaccessible to conventional astronomy. Information from even the gamma-ray burst is smoothed over by scattering within the matter that generated it, just as distance and dispersion transforms the clap of lightning to the low roar of thunder. Gravitational waves, by contrast, are scatter-proof, and carry the merger signature with better than 1 ms resolution. The authors model several different equations of state for the neutron star, and simulate the formation of the final black hole and associated disk for a wide range of parameters. They find, among other phenomena, that small spiral arms are formed around, and are eventually swallowed by, the black hole.
Disappointingly, the merger signal occurs at high frequencies, where detector sensitivity is limited by photon shot noise. At a distance of 100 Mpc, the peak strain amplitude for the equation of state used to generate Fig. 1 is 1ร10-22 at a frequency around 6 kHz, which is about a factor of 10 too weak to be seen by Advanced LIGO. According to the authors, a subsequent generation of detectors now in the conceptual planning stage, such as the Einstein Telescope,[15] will be needed to detect the merger. Then the theoretical calculations of the favorite source of ground-based detectors will finally be fully confronted with observational data.
Acknowledgment
The author thanks Curt Cutler for helpful discussions.
References
- 1. K. Kiuchi, Y. Sekiguchi, M. Shibata, and K. Taniguchi, Phys. Rev. Lett. 104, 141101 (2010).
- 2. J. Weber, Phys. Rev. 117, 306 (1960).
- 3. B. C. Barish and R. Weiss, Phys. Today 52, No. 10, 44 (1999).
- 4. P. L. Bender, K. Danzmann, and the LISA Study Team, "Laser Interferometer Space Antenna for the Detection of Gravitational Waves, Pre-Phase A Report," doc. MPQ 233, Max-Planck-Institรผt fรผr Quantenoptik, Garching, 1998.
- 5. Beyond Einstein Program Assessment Committee and National Research Council, NASA's Beyond Einstein Program: An Architecture for Implementation (National Academy Press, Washington, DC, 2007)[Amazon][WorldCat.]
- 6. R. E. Vogt, R. W. P. Drever, K. S. Thorne, and R. Weiss "Caltech/MIT project for a laser interferometer gravitational wave observatory," Renewal proposal for NSF PHY-8504136, December, 1987.
- 7. E. S. Phinney, Astrophys. J. Lett. 380, L17 (1991).
- 8. C. Cutler and K. S. Thorne, in Proceedings of the 16th International Conference on General Relativity and Gravitation, Durban, South Africa, 2001, edited by N. Bishop and S. D. Maharaj (World Scientific, Singapore, 2002)[Amazon][WorldCat.]
- 9. V. Kalogera et al., Astrophys. J. Lett. 603, L41 (2004).
- 10. D. Guetta and T. Piran, Astron. Astrophys 435, 421 (2005).
- 11. J. Abadie et al., arXiv:1003.2480.
- 12. E. Nakar, Phys. Rep. 442, 166 (2007); arXiv:astro-ph/0701748v2.
- 13. J. Abadie et al., arXiv:1001.0165.
- 14. http://www.ligo.caltech.edu/advLIGO/scripts/summary.shtml.
- 15. http://www.et-gw.eu/.
About the Author
Since 1997, Robert Spero has been an experimental physicist at JPL in Pasadena, California, where he contributes to the experimental and design effort to make the LISA mission a reality. Before that, he was a member of the LIGO group at Caltech from the earliest days, when all the members sat around a small table.
Reader Comments
to our Newsletter